Supply🔗
Supply of Extracted Fuels, Delivered Fuels, and Electricity Generation🔗
There are three main supply chains to capture the stock and flow of supply capacity of extracted fuel (coal, oil, gas, and biofuel), delivered fuel (coal, oil, gas, and biofuel), and electricity production from each of the electric paths (coal, oil, gas, biofuel, nuclear, hydro, wind, solar, geothermal, other renewables, and new). The model assumes that each extracted fuel is available only for its respective delivered fuel type. All fuels plus nuclear, hydro, renewable types, and new zero-carbon are available for the electric carrier.
Carriers | Sources Used |
---|---|
Coal Carrier | Coal |
Oil Carrier | Oil |
Gas Carrier | Gas |
Bio Carrier | Bio |
Electric Carrier | Elec Paths |
Each delivered fuel is available for nonelectric and electric use. The electric only paths are mapped to the primary source, where the renewable types are aggregated to Primary Renewables.
Primary Energy Sources | Primary Fuels | Elec Paths |
---|---|---|
Primary Coal | PCoal | ECoal |
Primary Oil | POil | EOil |
Primary Gas | PGas | EGas |
Primary Bio | PBio | EBio |
Primary Nuclear | Nuclear | |
Primary Hydro | Hydro | |
Primary Renewables | Wind Solar Geothermal Other Renewables |
|
Primary New | New |
Subscript ranges are grouped and mapped accordingly.
Range | Paths Included |
---|---|
Fossil Fuels | Coal: Primary Coal, PCoal, ECoal Oil: Primary Oil, POil, EOil Gas: Primary Gas, PGas, EGas |
Elec thermal | ECoal, EOil, EGas, EBio |
Electric Carrier | Elec thermal Nuclear Hydro Wind Solar Geothermal Other Renewables |
The capacity and utilization and cost of extracted fuels affect the market price of extracted fuels, which feeds into the variable cost of delivered fuels. As such, the market price of extracted fuels affects the capacity, utilization, and market price of delivered fuels, which in turn feeds into the variable cost of electric producers using those fuels. Accordingly, the costs of delivered fuels affect the capacity and utilization of those electricity sources. Market Clearing and Utilization details the market clearing and utilization of delivered fuels and electricity sources.
For each of these phases, the capacity represents the installed base of usable capital. It depreciates via a constant fractional rate, without age vintaging of the stock. The profitability, however, affects the rate of depreciation. For delivered fuels and electric supply, capacity must go through the development phase and then constructed before it can be used, introducing a delay between initiating and completing the acquisition of new capacity. The amount of capacity that is planned for construction accounts for the total capacity needed to meet the energy demand, including transmission and delivery losses, plus a reserve margin and expected growth of energy requirements.
For capacities of extracted fuels and of delivered fuels for nonelectric consumption, the desired capacity of each depends in part on the centralized effect of expected growth and normal utilization, as well as on the profitability and current capacity of each fuel.
Any non-cost policies banning new capacity adjust the resulting desired capacity.
For electric generation, the desired capacity of each source depends on the demand of electricity and the Fraction invested in elec energy source
, as well as the profitability and current capacity of each source.
Desired capacities are adjusted by dividing by the capacity factor of each resource, requiring more of each energy path to be constructed to get the actual desired supply.
The constructed supply is then multiplied by the capacity factor to yield the actual capacity.
While the Actual Supply Capacity represents the amount of energy from each path that can be dispatched, the Energy Supply Capacity is the amount of energy that is constructed.
The rate of capacity completion is constrained by the capacity to do so. This structure captures supply chain constraints, for example the fact that if wind turbine orders double overnight, completion of new turbines cannot also double immediately. It takes time to acquire labor and machinery and build up other aspects of the necessary supply chain. This has two consequences: with increasing pressure to construct capacity, the effective lead time increases, and the cost of new capacity rises.
Drivers of Cost of Supply🔗
Several factors affect the cost of each supply source, including,
- A baseline or reference cost
- a learning-by-doing effect from the accumulation of experience in capacity installation
- an exogenous user-specified cost reduction from technological breakthroughs achieved through research and development (R&D)
- cost of fuels as determined by the delivered fuel market price and efficiency of fuel use
- resource constraints
- source subsidies/taxes
- storage costs for variable renewables (solar and wind)
- soft costs for renewables
- emissions cost from carbon pricing
- qualifying electricity standards costs and penalties, Qualifying Electricity Standards (QES)
- a “pipeline overheating” premium from supply chain constraints on capacity installation, Supply of Extracted Fuels, Delivered Fuels, and Electricity Generation
Resource Constraints🔗
The Resource Constraints sector addresses the potential limits to available energy resources and the effects those limits may have on supply costs. The resource effect cost is a function of the depletion effect on cost and the supply curve effect on cost.
The depletion effect is dynamic, with cost increasing as cumulative production grows. This captures cost escalation with the depletion of fossil fuels. It is possible to discover unconventional resources, thereby reducing the depletion effect; however, it is assumed that the unconventional resources have a different carbon intensity, adjusted by the user. Biomass is not limited by depletion but rather by the supply constraints of each feedstock, i.e., wood, crops, and others, which reflects the limit of production of energy from a source from the saturation of production opportunities. These resource constraints affect the extraction costs of fuels, resulting in a greater market price of extracted fuels. In turn, a greater market price of extracted fuels drives up the market price of delivered fuels for nonelectric use and increases the cost to produce electricity from the thermal paths with and without CCS.
The supply curve constraint can also affect the cost to produce the electric only paths, i.e., nuclear, hydro, renewable types, and new zero-carbon; of these sources, the model defaults to only affect hydro and renewables. Supply limitations for these paths affect the O&M and capital costs, capturing, for example, the escalation in cost of wind power that occurs as the cheapest sites are exploited first.
Parameters are based on IPCC 2007 and IEA 2022 estimates.
Storage for Renewables🔗
Storage capacity for variable renewable energy (VRE) must meet its demand when the source is not available. Variable renewables include wind and solar, whereas geothermal and other renewables are more constant in their generation. Models of hourly, daily, and seasonal variability of demand and renewable generation determined the storage coverage, i.e., energy per variable renewable capacity (EJ per EJ/year), and the average power needed from storage per variable renewable capacity (EJ/year per EJ/year). Sensitivity analyses of each category of coverage determined the relationships between these parameters and the share of VRE capacity to total electric generation capacity. These analyses also confirmed the effect that round trip efficiency (RTE) has on the required coverage; it scales 1/RTE such that the lower the RTE to use storage, the higher the maximum storage capacity for storage required. The resulting relatingship between hours of coverage versus VRE share is consistent with that found by others, including Solomon et al. (2017), Shaner et al. (2018), and Albertus (2020).
The sum of coverages for the different categories of variability is allocated to short (<4 hours), medium (4-12 hours), and long duration storage (>12 hours). Besides storage, other demand response technology, long-distance transmission, and behavioral load management can minimize storage needs but only for short and medium coverage. Learning and investment increases the percent effect these options have on the storage requirements.
While the model assumes that storage requirements will not limit utilization, costs of renewables account for those for storage. Comparable to the experience and breakthrough effects for energy, storage costs also decrease with cumulative capacity installation and potential technological breakthroughs.
Storage could be in the form of batteries, compressed air, pumped hydropower, and other more novel options, including hydrogen. NREL's Store-FAST: Energy Storage Financial Analysis Scenario Tool, version: 1.2 (2019), provides the inputs for power and energy costs, including RTE, for several storage options. The levelized cost of each type adds the cost of electricity for it, which is the market price of electricity divided by the storage RTE.
Other than hydrogen, these technologies all become limited and/or more costly with longer durations of coverage. Cost effective long duration coverage is critical when the share of VRE exceeds approximately 70-80%. Hydrogen, despite having a much lower RTE, approximately 36%, has the potential to provide more cost effective long duration coverage than the other technologies. This is because, although the power costs associated with hydrogen far exceed those other storage options, the costs to store each hour of energy coverage is far less than the other options. Despite that cost effectiveness for long-term coverage, ancillary costs currently associated with hydrogen storage constrain its use. A breakthrough in hydrogen also reduces these ancillary costs, thereby allowing it to take advantage of its cost attractiveness for long-term coverage.
Electricity for Storage🔗
In addition to the power and energy costs, each storage option also requires electricity for charging and discharging. Electricity exceeding that which is generated from storage, determined from the sum of the average power needed from storage per variable renewable capacity from the hourly, daily, and seasonal models, is added to the industrial electric carrier demand. For example, an RTE of 100% requires no additional electricity; an RTE of 80% requires 0.25 times the power required; and an RTE of 36% requires 1.77 times the power required. By default, the electricity all comes from the grid.
Hydrogen Leakage🔗
Hydrogen leakage, defaulted at 2%/year, releases hydrogen to the atmosphere. While there is no direct radiative forcing from hydrogen, the climate structure accounts for its indirect effects on the radiative forcings of CH4, O2, H2O, and aerosols (Sand et al., 2023).
Soft Costs and Subsidies for Renewables🔗
The levelized cost of electricity (LCOE) of renewables, particularly wind and solar energy, have decreased dramatically since 1990, especially over the past decade. Two opposing forces have contributed to those declines with the energy generated by them. There have been historical subsidies for solar and wind, defined as a fraction of their direct costs, stimulating their growth. While the fraction of solar subsidies declined over time until 2020, that fraction and the fraction for wind is expected to remain constant through 2100 as Baseline subsidies, comparable to the fossil fuel subsidies embedded in their costs. However, the user can end them sooner. There have also been soft costs, i.e., indirect costs, that have made the investment in these sources less attractive than direct cost alone would suggest. It captures the soft costs as an initial level that declines with experience at a rate determined by a progress ratio. The values defining these subsidies and soft costs were estimated from literature and set through optimization to fit historical cost (IRENA, 2020; Lazard, 2021; IEA, 2020) and energy data (IEA, 2022; BP, 2022).
Sources of LCOE data for renewables are not consistently presented and only available for some years, therefore requiring conversions and bridging between datasets.
IRENA: All LCOE results are reported in $2019 USD. Reported values calculated excluding any financial support and using a fixed assumption of a real cost of capital of 7.5% in OECD countries and China, and 10% in the rest of the world, unless explicitly mentioned. All LCOE calculations exclude the impact of any financial support. Converted to $2017.
LAZARD 3.0-15.0: All LCOE results reported in nominal dollars. Each analysis assumes 60% debt at 8% interest rate and 40% equity at 12% cost; Unless otherwise indicated, the analysis herein does not reflect decommissioning costs, ongoing maintenance-related capital expenditures or the potential economic impacts of federal loan guarantees or other subsidies; Lazard’s unsubsidized LCOE analysis indicates significant historical cost declines for utility-scale renewable energy generation technologies. Converted to $2017.
IEA Levelized Costs Data: Global average LCOEs and auction results for utility-scale PV by commissioning date. Last updated 26 Oct 2022. Data shown = LCOE in $2017.
IEA: Evolution of solar PV module cost by data source, 1970-2020. Last updated 26 Oct 2022. While the LCOE data for solar PV is not readily available before 2009, IEA’s cost per watt of solar PV from IEA 1970-2020 provides data to estimate the LCOE from 1990. Using the ratio of annual costs per watt to that in 2010 and applying that ratio to the IRENA solar PV LCOE in 2010 provides an estimate of LCOE from 1990-2019.
Berkeley. Median 30-Year LCOE without the ITC reported in $2018. Converted to $2017.
Utility vs distributed solar PV: There are differences between utility scale and distributed solar PV. According to IEA (2022), the fraction of PV that is utility scale grew from 24% to 50% of solar PV between 2010-2016, remaining at that level thereafter. Lazard provides utility scale and distributed cost data; accordingly, comparisons are made to the weighted average of these. The weights assume the trend of increasing utility scale relative to distributed increases at a comparable rate to history.
Onshore vs offshore wind: Likewise, there are differences between onshore and offshore wind. IRENA used the weighted average of the onshore fraction of wind, taken from IEA Wind Electricity Report, to get the weighted average of wind LCOE. From regional graphs of onshore vs offshore wind, they estimated wind to be 100% onshore until 2010, when offshore wind starts to present, decreasing down to 95% by 2019.
Instant and Embodied Supply Costs and Efficiencies🔗
The embodied costs of supply are modeled in the Embodied Supply Costs sector. These costs factor into the utilization of energy capacity in the Market Clearing and Utilization sector. Embodied costs represent the actual physically-imposed costs, which are locked in at the time of capacity investment, i.e., new capacity development. Variable costs include operation and maintenance (O&M), and fuel costs. The fuel costs for delivered fuels are the extracted fuel costs. The fuel cost for each electric source requiring fuel is the market price of extracted fuel, accounting for a markup, divided by the embodied thermal efficiency of the source; fuel prices for the primary electric paths are 0. Unit profit, which is the revenue less the variable costs, may be adjusted by a tax/subsidy and/or carbon tax to the producers of delivered fuels.
As in the Demand sector, the construction pipeline is explicit but without vintaging of capital as there is in the demand side; costs are assumed to be well-mixed. All inputs to this sub-model are determined in other sub-models except for the Overheating cost sensitivity, which is set at 0.5. The embodied costs and efficiencies of supply are locked in at the time new capacity development.
The effects on costs apply to the levelized capital costs as well as to O&M and fuel costs.
Electric Supply Choice🔗
As in the Carrier Choice sectors, the fraction of new investment allocated to each of the electric energy sources is a function of its attractiveness relative to that of the other sources. Attractiveness synthesizes cost effect and the effect of a performance standard.
The cost, adjusted by any source subsidies/taxes, drives the cost attractiveness of each electric path relative to the other electric paths.
The performance standard effect is a function of a specified carbon intensity threshold and the carbon intensity of each energy source resource, defined in Emissions. The performance standard creates a soft threshold, beyond which sources with high emissions intensity (e.g., coal) are greatly diminished in attractiveness and are effectively eliminated from the investment mix. The effect of non-cost policies aims to capture any legislation or rule demanding no new investment in a specified source for a percentage of the global energy needs.
Model Structure🔗
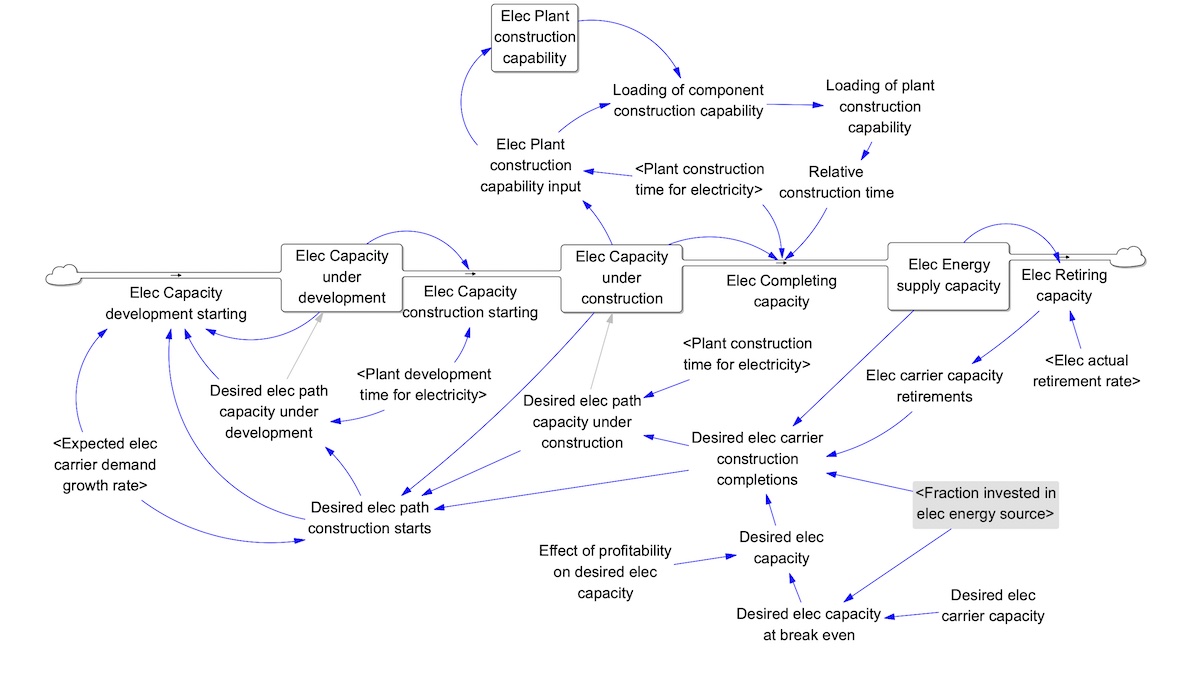